Development of novel catalyst materials of CO2 Utilization
Introduction
As greenhouse gas emissions are on a steady rise the need for catalyst materials that can convert these gases into something useful is arising as well. Most conventional catalysts however, suffer from severe deactivation at temperatures needed to activate CO2. Therefore, our research focuses on a novel material class called perovskite-type oxides that shows promising properties that negate this deactivation effects.
Conventional catalysts used for CO2 utilization often consist of an oxide support material with metallic nanoparticles deposited on the surface. These nanoparticles are only loosely bonded to the surface which means they can move around and, especially at elevated temperatures, sinter together leading to a loss in active surface area and consequently a decrease in catalytic activity. With perovskite-type oxides we are able to grow this highly active nanoparticles out of the host oxide. This leads to a tighter bond between the formed nanoparticles and the pristine oxide and prevents sinter processes. Additionally, the rich oxygen chemistry of perovskite-type oxides is countering coking, another prominent process in catalyst deactivation.
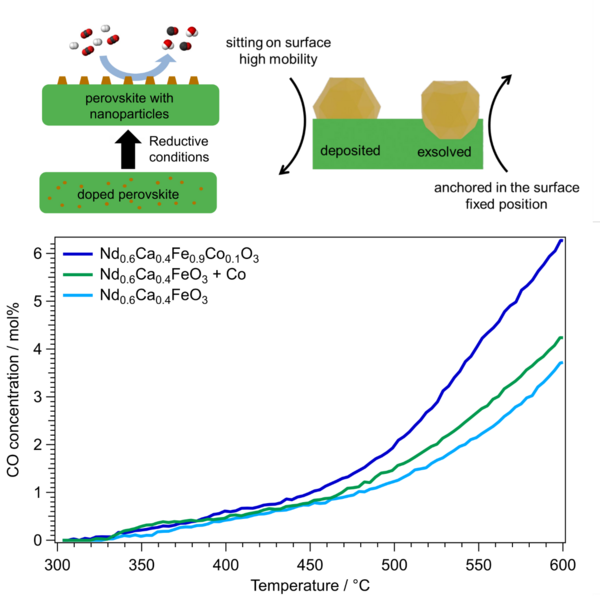
Approach
We us an intelligent design approach to tune our perovskite oxide catalysts to their specific task. This means we first try to understand the effects at play during the reaction and then adjust the catalyst in a controlled way to try to counter possible deactivation effects and improve activating effects. For this fundamental understanding we employ a wide range of techniques.
Catalytic tests (top left in Figure 2) are necessary to assess if the implemented changes to the catalyst are working for the desired reaction. Theoretical modeling using Density Functional Theory (DFT, top right in Figure 2) is used to understand the fundamental chemical changes the catalyst undergoes if new or different elements are doped into them. Characterization of the catalyst after the reaction is an important tool to investigate the changes the catalyst undergoes during the reaction. The most common techniques are electron microscopy (SEM, bottom left in Figure 2) and X-ray diffraction. This allows us to assess the emergence of possible deactivating products on the catalyst and if the structure of the material itself is stable. But also, the characterization of the catalyst during the reaction, referred to as in-situ or operando, is of uttermost importance. Only with techniques such as in-situ X-ray diffraction (bottom right in Figure 2) and in-situ X-ray photoelectron spectroscopy (XPS) it is possible to observe the catalyst during operating conditions. A combination of the knowledge gained from all these techniques let us improve our catalysts systematically.
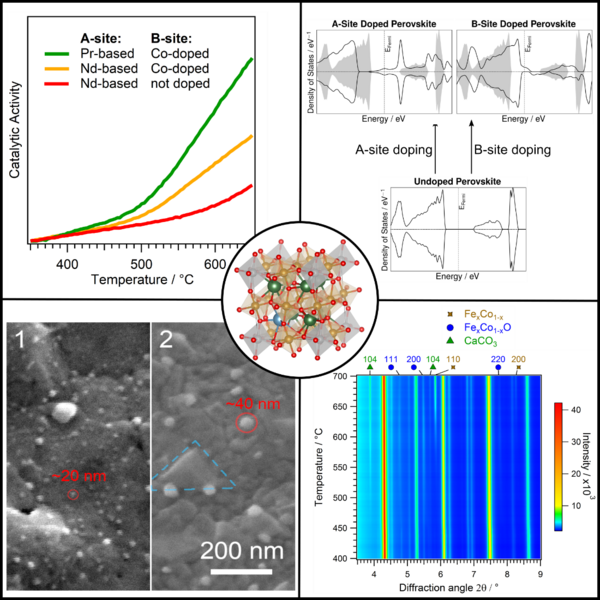
Results
We were already able to tune catalyst for CO2 reduction with H2 (rWGS reaction) and CH4 (dry reforming of methane). However, further improvement of the materials is still possible as there are still some deactivating phases present under reaction conditions. So, life is good but it could be better.
An open point we are currently working on is how to tune the exsolution process to control the formation of catalytic active nanoparticles. As the exsolution process itself is not yet fully understood by the community the tuning of the size and amount of the produced nanoparticles still poses some challenges. Furthermore, we have found that the exsolution of bigger nanoparticles actually enables unwanted sintering effects again as the distance between the particles is decreased substantially (Figure 3). This makes control over the exsolution process an absolute necessity to guarantee the long-term stability of perovskite-type oxide catalyst.
Strategies to better control the exsolution process are to use different compositions of the catalyst, tune the dopant amount or use different methods of exsolution such as a reductive electrical bias.
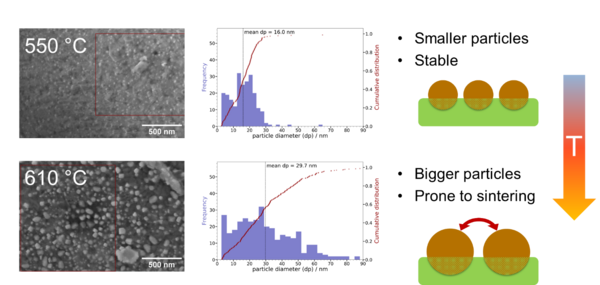